The Solid State
Ionic Solids:
Ionic solids can be defined as:
The crystalline solids which are formed by neatly packed ions of opposite charge. Ionic compounds are usually formed when metals react with non-metals.
In other words, ionic compounds are held together by ionic bonds are classed as ionic compounds. Elements can gain or lose electrons in order to attain their nearest noble gas configuration. The formation of ions (either by gaining or losing electrons) for the completion of octet helps them gain stability.
In a reaction between metals and non-metals, metals generally loose electrons to complete their octet while non-metals gain electrons to complete their octet. Metals and non-metals generally react to form ionic compounds.
Ionic Compound Structure:
The structure of an ionic compound depends on the relative sizes of the cations and anions. Ionic compounds include salts, oxides, hydroxides, sulphides, and the majority of inorganic compounds. Ionic solids are held together by the electrostatic attraction between the positive and negative ions.
For example, the sodium ions attract chloride ions and the chloride ion attracts sodium ions. The result is a three-dimensional structure of alternate Na+ and Cl– ions. This is a crystal of sodium chloride. The crystal is uncharged because the number of sodium ions is equal to the number of chloride ions. The forces of attraction between the ions hold them in the structures.
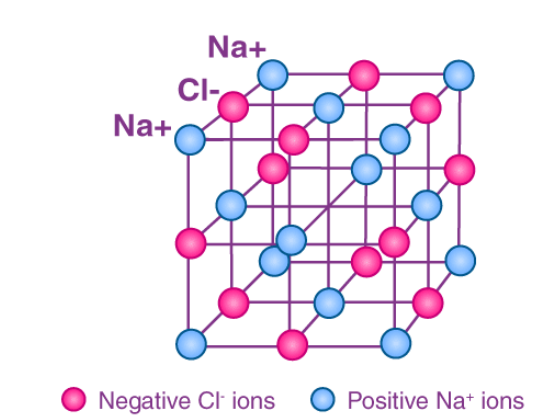
These ionic bonds between the charged particles result in a giant structure of ions. Because the ions are held together tightly in these giant structures it takes a lot of energy to break all the bonds. As a result, ionic compounds have high melting points and boiling points.
Example - The reaction between magnesium and chlorine. The magnesium atom has two electrons in its outermost shell. By losing two electrons from its M shell its L shell becomes the outermost shell that has a stable octet. The nucleus of this magnesium atom still has twelve protons but the number of electrons has decreased to ten. So, a net positive charge is developed on this magnesium atom, giving a magnesium cation Mg2+.
On the other hand, the chlorine atom has seven electrons in its outermost shell. Therefore, it needs only one electron to complete its octet. It can gain this one electron from the electrons lost by the magnesium atom to become magnesium ion. As two electrons are lost by a magnesium atom while one chlorine atom can gain only one electron, two atoms of chlorine combine with one atom of magnesium to form magnesium chloride.
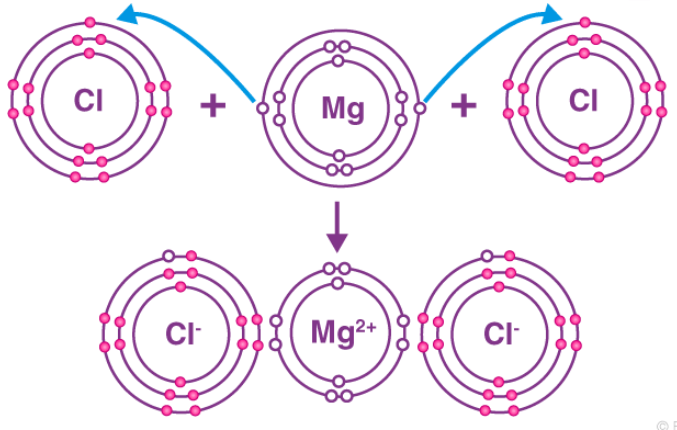
From the above example, ionic compounds can be defined as the compounds formed by the transfer of electrons between metals and non-metals. The bond formed between them is known as the ionic bond. Due to the presence of oppositely charged ions, ionic compounds are held strongly by the electrostatic force of attraction.
Ionic Compound Properties:
1. Physical properties of ionic compounds
Due to the presence of the strong force of attraction between the positive and negative ions, ionic compounds are solids and are hard to break. They generally break into pieces when pressure is applied, hence they are considered brittle.
2. Melting and boiling points of ionic compounds
Due to the presence of electrostatic forces of attraction between ions, a large amount of energy is required to break the ionic bonds between the atoms. Thus, ionic compounds have high melting and boiling points.
3. The solubility of ionic compounds
Ionic compounds are generally soluble in polar solvents such as water whereas solubility tends to decrease in non-polar solvents such as petrol, gasoline, etc.
4. Conduction of Electricity
Ionic compounds do not conduct electricity in the solid-state but are good conductors in a molten state. Conduction of electricity involves the flow of charge from one point to another. In the solid-state, as the movement of ions is not possible, ionic compounds don’t conduct electricity. Whereas in the molten state, ionic compounds conduct electricity as electrostatic forces of attraction between the ions are overcome by the heat released.
Metallic Bonds and Metallic Solids:
‘Metallic bond’ is a term used to describe the collective sharing of a sea of valence electrons between several positively charged metal ions.
Metallic bonding is a type of chemical bonding and is responsible for several characteristic properties of metals such as their shiny lustre, their malleability, and their conductivities for heat and electricity.
Both metallic and covalent bonding can be observed in some metal samples. For example, covalently bonded gallium atoms tend to form crystal structures that are held together via metallic bonds. The mercurous ion also exhibits metallic and covalent bonding.
The factors that affect the strength of a metallic bond include:
- Total number of delocalized electrons.
- Magnitude of positive charge held by the metal cation.
- Ionic radius of the cation
An illustration describing the way electrons are delocalized over a rigid lattice of metal ions in a metallic bond is provided below.
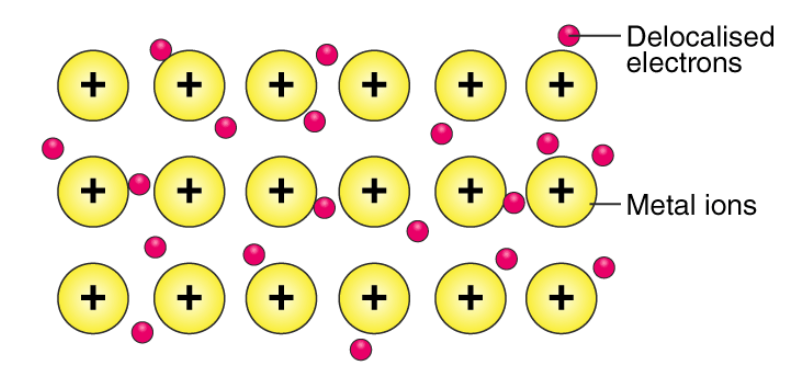
Metallic bonds are not broken when the metal is heated into the melt state. Instead, these bonds are weakened, causing the ordered array of metal ions to lose their definite, rigid structure and become liquid. However, these bonds are completely broken when the metal is heated to its boiling point.
Example - The electron configuration of sodium is 1s22s22p63s1; it contains one electron in its valence shell. In the solid-state, metallic sodium features an array of Na+ ions that are surrounded by a sea of 3s electrons. However, it would be incorrect to think of metallic sodium as an ion since the sea of electrons is shared by all the sodium cations, quenching the positive charge.
An illustration describing the metallic bonding in sodium is provided below.
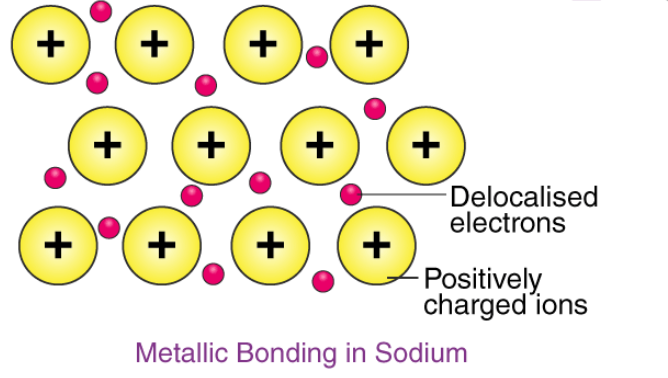
The softness and low melting point of sodium can be explained by the relatively low number of electrons in the electron sea and the relatively small charge on the sodium cation. For example, metallic magnesium consists of an array of Mg2+ ions. The electron sea here contains twice the number of electrons than the one in sodium (since two 3s electrons are delocalized into the sea). Due to the greater magnitude of charge and the greater electron density in the sea, the melting point of magnesium (~650oC) is significantly higher than that of sodium.
Properties Attributed by Metallic Bonding:
Metallic bonds impart several important properties to metals that make them commercially desirable. Some of these properties are briefly described in this subsection.
1. Electrical Conductivity
Electrical conductivity is a measure of the ability of a substance to allow a charge to move through it. Since the movement of electrons is not restricted in the electron sea, any electric current passed through the metal passes through it, as illustrated below.
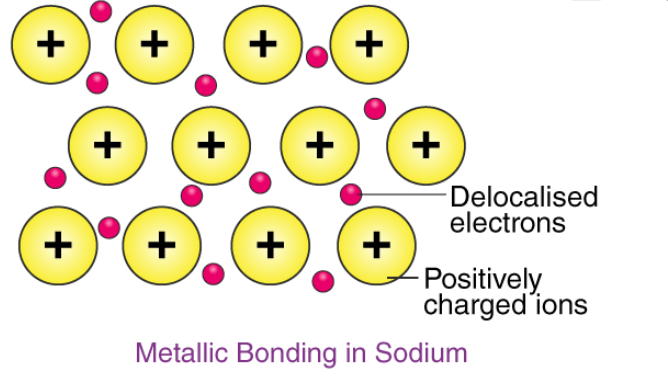
When a potential difference is introduced to the metal, the delocalized electrons start moving towards the positive charge. This is the reason why metals are generally good conductors of electric current.
2. Thermal Conductivity
The thermal conductivity of a material is a measure of its ability to conduct/transfer heat. When one end of a metallic substance is heated, the kinetic energy of the electrons in that area increases. These electrons transfer their kinetic energies to other electrons in the sea via collisions.
The greater the mobility of the electrons, the quicker the transfer of kinetic energy. Due to metallic bonds, the delocalized electrons are highly mobile, and they transfer the heat through the metallic substance by colliding with other electrons.
3. Malleability and Ductility
When an ionic crystal (such as sodium chloride crystal) is beaten with a hammer, it shatters into many smaller pieces. This is because the atoms in the crystals are held together in a rigid lattice that is not easily deformed. The introduction of a force (from the hammer) causes the crystal structure to fracture, resulting in the shattering of the crystal.
In the case of metals, the sea of electrons in the metallic bond enables the deformation of the lattice. Therefore, when metals are beaten with a hammer, the rigid lattice is deformed and not fractured. This is why metals can be beaten into thin sheets. Since these lattices do not fracture easily, metals are said to be highly ductile.
4. Metallic Luster
When light is incident on a metallic surface, the energy of the photon is absorbed by the sea of electrons that constitute the metallic bond. The absorption of energy excites the electrons, increasing their energy levels. These excited electrons quickly return to their ground states, emitting light in the process. This emission of light due to the de-excitation of electrons attributes a shiny metallic lustre to the metal.
5. High Melting and Boiling Points
As a result of powerful metallic bonding, the attractive force between the metal atoms is quite strong. In order to overcome this force of attraction, a great deal of energy is required. This is the reason why metals tend to have high melting and boiling points. The exceptions to this include zinc, cadmium, and mercury (explained by their electron configurations, which end with ns2).
The metallic bond can retain its strength even when the metal is in its melt state. For example, gallium melts at 29.76oC but boils only at 2400oC. Therefore, molten gallium is a non volatile liquid.
Molecular Solids:
Molecular solids are one type of Crystalline solid. It is pertinent to the London forces and intermolecular forces that are held between two chlorine molecules. It is relevant to the polar covalent bond. In various Crystalline solids types, it is one of the constituent particles of the Molecular solid that is packed up jointly. They are molecules of the substance. All these arranged molecules can be non–polar, which has the dipole moment = 0, etc., or they may be polar has dipole moment > 0, etc.
Moreover, it is made up of molecules and atoms, and it is made up together with van der Waals forces. Because of this dipole, London forces are then weaker from the iconic and covalent bond. Ordinarily, the molecular solids are essentially too smooth and have a moderately very low melting temperature. Apart from this, there are molecular solids classified into three types: non-polar molecular solids, Polar molecular solids, and Hydrogen-bonded molecular solids. For instance, HCl, F, O, N, etc.
WHAT IS LONDON DISPERSION FORCE?:
The London force is excessively too weak attractive forces among molecules and atoms. This force is essentially caused by electrostatic attraction. Moreover, the London forces are the most attractive forces that induce due to the nonpolar substances. It is caused due to reduction of freezing and liquids into solids. It is based upon the temperature being reduced adequately.
Types of Molecular Solids:
i) Polar Molecular solids:
It is held with molecules and atoms by a polar covalent bond. Also, the atoms and molecules are communed by relatively more robust dipole interactions. Also, the properties of this are that the physical nature of the molecules is too smooth and which includes almost all liquids and gases, it is based upon the room temperature. The Polar Molecular solids have not conducted electricity, and it has a higher melting point as compared to the non-polar molecular solids. Examples of this are HCl, SO2, NH3, etc. So, that’s all about the first type of molecular solids, which is polar molecular solids.
(ii) Non-Polar Molecular solids:
Instead of the Polar Molecular solids, then another type of Molecular solids is non-molecular solids. The molecules are formed with Molecules and atoms. It’s relatively shared together with a nonpolar covalent bond. Apart from this, the atoms and molecules are basically held by too weak London forces and dispersion forces. The properties of the Non Molecular solids are that the physical nature of the Non Molecular solids is too soft as compared to polar molecular solids. Moreover, the Non Molecular solids are not able to conduct electricity. Hence they are known as an insulator. The melting point of the Non Molecular solids is too low compared to molecular types. An example of the Non Molecular solids are H2, Cl2, I2, etc.
(iii) Hydrogen Molecular Solids:
Another type of Molecular solid is Hydrogen-Bonded Molecular Solids, which have included the polar covalent bonds with Oxygen, nitrogen, hydrogen, and other Fluorine atoms. The Hydrogen-Bonded Molecular Solids are maintained jointly by the most powerful hydrogen bonding. Apart from this, the property of the Hydrogen-Bonded Molecular Solids is that their physical nature is too hard as compared to its other types. The Hydrogen-Bonded Molecular Solids are not able to conduct electricity. Despite this, the physical state of the Hydrogen-Bonded Molecular Solids is exclusively too soft and volatile liquids solids as per or under the room temperature. Furthermore, the Hydrogen-Bonded Molecular Solids melting point is too low. Examples of the Hydrogen-Bonded Molecular Solids are ice or H2O.
Covalent Network Solids:
Covalent solids are formed by networks or chains of atoms or molecules held together by covalent bonds. A perfect single crystal of a covalent solid is therefore a single giant molecule. For example, the structure of diamond, shown in part (a) in the figure below, consists of sp3 hybridized carbon atoms, each bonded to four other carbon atoms in a tetrahedral array to create a giant network. The carbon atoms form six-membered rings.

(a) Diamond consists of sp3 hybridized carbon atoms, each bonded to four other carbon atoms. The tetrahedral array forms a giant network in which carbon atoms form six-membered rings. This is easier to see in the rotating cube shown in the figure below.
(b) These side (left) and top (right) views of the graphite structure show the layers of fused six-membered rings and the arrangement of atoms in alternate layers of graphite. The rings in alternate layers are staggered, such that every other carbon atom in one layer lies directly under (and above) the center of a six-membered ring in an adjacent layer.
All compounds with the diamond and related structures are hard, high-melting-point solids that are not easily deformed. Instead, they tend to shatter when subjected to large stresses, and they usually do not conduct electricity very well. In fact, diamond (melting point = 3500°C at 63.5 atm) is one of the hardest substances known, and silicon carbide (melting point = 2986°C) is used commercially as an abrasive in sandpaper and grinding wheels. It is difficult to deform or melt these and related compounds because strong covalent (C–C or Si–Si) or polar covalent (Si–C or Si–O) bonds must be broken, which requires a large input of energy.
Other covalent solids have very different structures. For example, graphite, the other common allotrope of carbon, has the structure shown in part (b) in the figure above. It contains planar networks of six-membered rings of sp2 hybridized carbon atoms in which each carbon is bonded to three others. This leaves a single electron in an unhybridized 2pz orbital that can be used to form C=C double bonds, resulting in a ring with alternating double and single bonds. Because of its resonance structures, the bonding in graphite is best viewed as consisting of a network of C–C single bonds with one-third of a π bond holding the carbons together, similar to the bonding in benzene.
The C–C distance in graphite (141.5 pm) is slightly longer than the distance in benzene (139.5 pm), consistent with a net carbon–carbon bond order of 1.33. In graphite, the two-dimensional planes of carbon atoms are stacked to form a three-dimensional solid; only London dispersion forces hold the layers together. As a result, graphite exhibits properties typical of both covalent and molecular solids. Due to strong covalent bonding within the layers, graphite has a very high melting point, as expected for a covalent solid (it actually sublimes at about 3915°C). It is also very soft; the layers can easily slide past one another because of the weak interlayer interactions. Consequently, graphite is used as a lubricant and as the “lead” in pencils; the friction between graphite and a piece of paper is sufficient to leave a thin layer of carbon on the paper. Graphite is unusual among covalent solids in that its electrical conductivity is very high parallel to the planes of carbon atoms because of delocalized C–C π bonding. Finally, graphite is black because it contains an immense number of alternating double bonds, which results in a very small energy difference between the individual molecular orbitals. Thus light of virtually all wavelengths is absorbed. Diamond, on the other hand, is colorless when pure because it has no delocalized electrons.
Diamond:
The structure of diamond is shown at the right in a "ball-and-stick" format. The balls represent the carbon atoms and the sticks represent a covalent bond. Be aware that in the "ball-and-stick" representation the size of the balls do not accurately represent the size of carbon atoms. In addition, a single stick is drawn to represent a covalent bond irrespective of whether the bond is a single, double, or triple bond or requires resonance structures to represent. In the diamond structure, all bonds are single covalent bonds (σ bonds). The "space-filling" format is an alternate representation that displays atoms as spheres with a radius equal to the van der Waals radius, thus providing a better sense of the size of the atoms.

Notice that diamond is a network solid. The entire solid is an "endless" repetition of carbon atoms bonded to each other by covalent bonds. (In the display at the right, the structure is truncated to fit in the display area.)
Graphite:
The most stable form of carbon is graphite. Graphite consists of sheets of carbon atoms covalently bonded together. These sheets are then stacked to form graphite. The figure below shows a ball-and-stick representation of graphite with sheets that extended "indefinitely" in the xy plane, but the structure has been truncated for display purposed. Graphite may also be regarded as a network solid, even though there is no bonding in the z direction. Each layer, however, is an "endless" bonded network of carbon atoms.

Fullerene:
The existence of C60, which resembles a soccer ball, had been hypothesized by theoreticians for many years. In the late 1980's synthetic methods were developed for the synthesis of C60, and the ready availability of this form of carbon led to extensive research into its properties.


The C60 molecule , is called buckminsterfullerene, though the shorter name fullerene is often used. As is evident from the display, C60 is a sphere composed of six-member and five-member carbon rings. These balls are sometimes fondly referred to as "Bucky balls". It should be noted that fullerenes are an entire class of pure carbon compounds rather than a single compound. A distorted sphere containing more than 60 carbon atoms have also been found, and it is also possible to create long tubes. All of these substances are pure carbon.
What is a Crystal Structure?:
A crystal structure is made of atoms. A crystal lattice is made of points. A crystal system is a set of axes. In other words, the structure is an ordered array of atoms, ions or molecules.
Crystal Structure is obtained by attaching atoms, groups of atoms or molecules. This structure occurs from the intrinsic nature of the constituent particles to produce symmetric patterns. A small group of a repeating pattern of the atomic structure is known as the unit cell of the structure. A unit cell is the building block of the crystal structure and it also explains in detail the entire crystal structure and symmetry with the atom positions along with its principal axes. The length, edges of principal axes and the angle between the unit cells are called lattice constants or lattice parameters.
Unit Cell:
Crystals use x-rays, which excite signals from the atom. The signals given by these atoms have different strengths, and they usually depend upon the electron density distribution in closed shells. The signals released by the atoms varies. Lighter the atoms, weaker is their signals. The mutual arrangement of atoms is also known as crystal structures. Crystal structures are derived from the physical density and chemical formulas of solids.
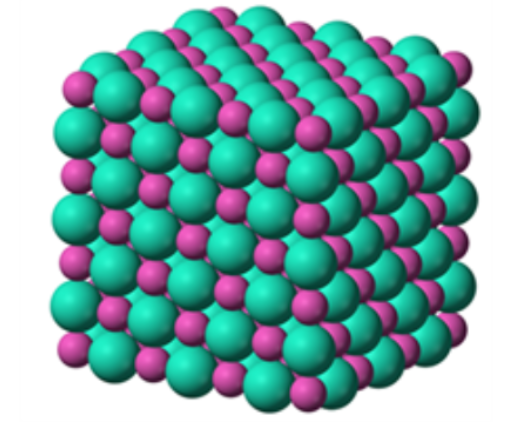
Unit cell can be defined as the smallest part of a component of the crystal. A group of atoms, ions or molecules, which are arranged together in a pure manner to build the crystal. The unit cells are structured in three-dimensional space, which describes the bulk arrangement of atoms of the crystal.
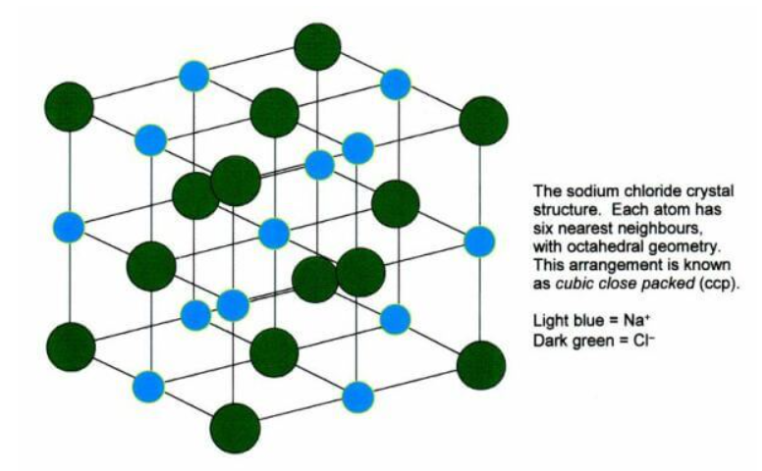
Crystal Systems:
A Crystal System refers to one of the many classes of crystals, space groups, and lattices. In crystallography terms, lattice system and crystal, the system are associated with each other with a slight difference. Based on their point groups crystals and space groups are divided into seven crystal systems.
The Seven Crystal Systems is an approach for classification depending upon their lattice and atomic structure. The atomic lattice is a series of atoms that are organized in a symmetrical pattern. With the help of the lattice, it is possible to determine the appearance and physical properties of the stone. It is possible to identify which crystal system they belong to. In a Cubic System crystals are said to represent the element earth. They are Seven Crystal Systems and are stated below with illustrated examples:
Triclinic System:
It is the most unsymmetrical crystal system. All three axes are inclined towards each other, and they are of the same length. Based on the three inclined angles the various forms of crystals are in the paired faces. Some standard Triclinic Systems include Labradorite, Amazonite, Kyanite, Rhodonite, Aventurine Feldspar, and Turquoise.
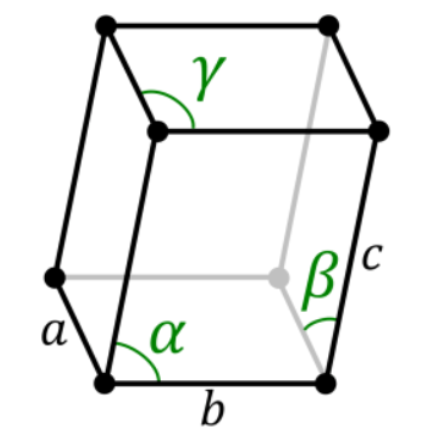
Monoclinic System
It comprises three axes where two are at right angles to each other, and the third axis is inclined. All three axes are of different length. Based on the inner structure the monoclinic system includes Basal pinacoids and prisms with inclined end faces. Some examples include Diopside, Petalite, Kunzite, Gypsum, Hiddenite, Howlite, Vivianite and more.
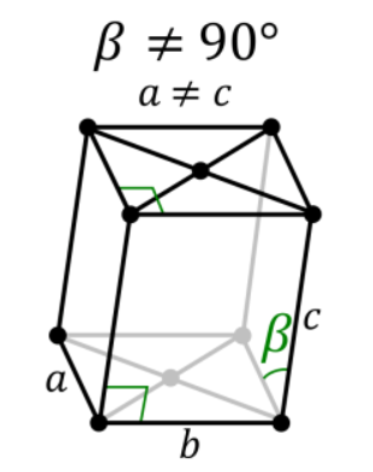
Orthorhombic System:
It comprises three axes and is at right angles to each other. There are different lengths. Based on their Rhombic structure the orthorhombic system includes various crystal shapes namely pyramids, double pyramids, rhombic pyramids, and pinacoids. Some common orthorhombic crystals include Topaz, Tanzanite, Iolite, Zoisite, Danburite and more.
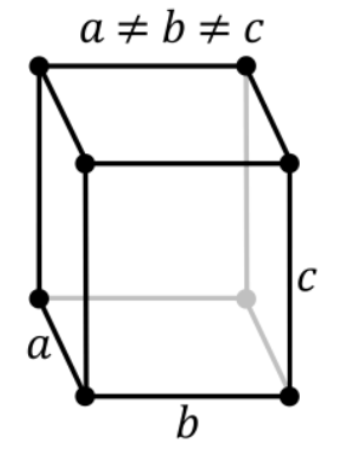
Trigonal System
Angles and axis in a trigonal system are similar to Hexagonal Systems. At the base of a hexagonal system (ross-section of a prism), there will be six sides. In the trigonal system (base cross-section) there will be three sides. Crystal shapes in a trigonal system include three-sided pyramids, Scalenohedral and Rhombohedra. Some typical examples include Ruby, Quartz, Calcite, Agate, Jasper, Tiger’s Eyes and more.
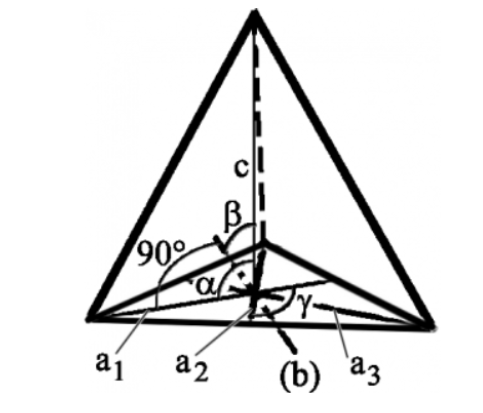
Hexagonal System:
It comprises four axes. The three a1, a2 and a3 axes are all contained within a single plane (called the basal plane) and are at 120°. They intersect each other at an angle of sixty degrees. The fourth axis intersects other axes at right angles. Crystal shapes of hexagonal systems include Double Pyramids, Double-Sided Pyramids, and Four-Sided Pyramids. Example: Beryl, Cancrinite, Apatite, Sugilite, etc.
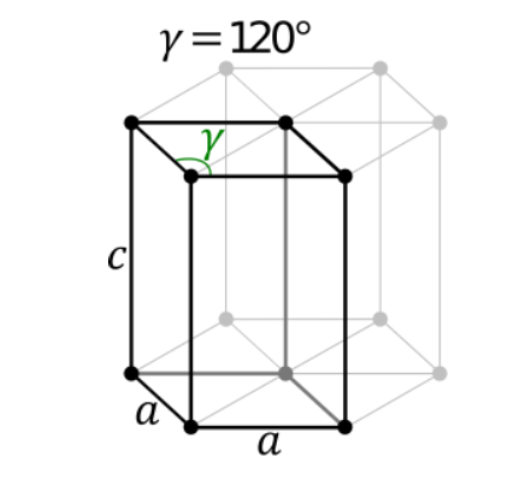
Tetragonal System:
It consists of three axes. The main axis varies in length; it can either be short or long. The two-axis lie in the same plane and are of the same length. Based on the rectangular inner structure the shapes of crystal in tetragonal include double and eight-sided pyramids, four-sided prism, trapezohedrons, and pyrite.
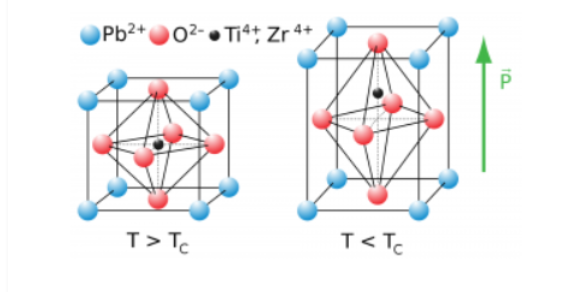
Cubic System:
Cubic system is the most symmetrical one out of the seven crystal system. All three angles intersect at right angles and are of equal length. Crystal shapes of a cubic system based on inner structure (square) include octahedron, cube, and Hexaciscohedron. Example: Silver, Garnet, Gold, and Diamond.
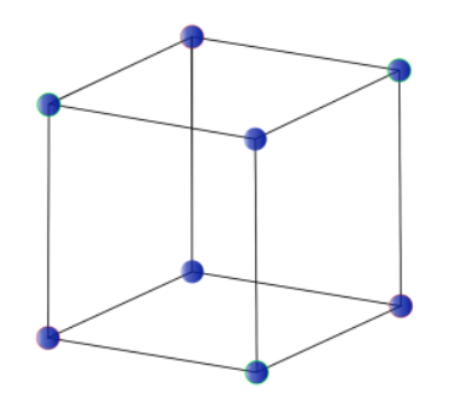
Bravais Lattices:
Bravais Lattice refers to the 14 different 3-dimensional configurations into which atoms can be arranged in crystals. The smallest group of symmetrically aligned atoms which can be repeated in an array to make up the entire crystal is called a unit cell.
There are several ways to describe a lattice. The most fundamental description is known as the Bravais lattice. In words, a Bravais lattice is an array of discrete points with an arrangement and orientation that look exactly the same from any of the discrete points, that is the lattice points are indistinguishable from one another.
Thus, a Bravais lattice can refer to one of the 14 different types of unit cells that a crystal structure can be made up of.
Types of Bravais Lattices:
Out of 14 types of Bravais lattices some 7 types of Bravais lattices in three-dimensional space are listed in this subsection. Note that the letters a, b, and c have been used to denote the dimensions of the unit cells whereas the letters 𝛂, 𝞫, and 𝝲 denote the corresponding angles in the unit cells.
1. Cubic Systems:
In Bravais lattices with cubic systems, the following relationships can be observed.
a = b = c
𝛂 = 𝞫 = 𝝲 = 90o
The 3 possible types of cubic cells have been illustrated below:
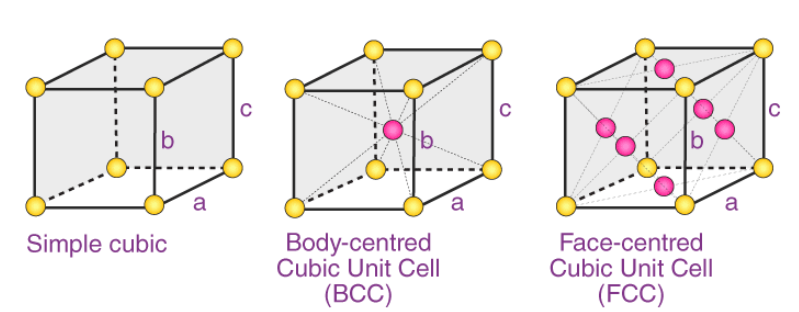
These three possible cubic Bravais lattices are –
- Primitive (or Simple) Cubic Cell (P)
- Body-Centered Cubic Cell (I)
- Face-Centered Cubic Cell (F)
Example: Polonium has a simple cubic structure, iron has a body-centered cubic structure, and copper has a face-centered cubic structure.
2. Orthorhombic Systems
The Bravais lattices with orthorhombic systems obey the following equations:
a ≠ b ≠ c
𝛂 = 𝞫 = 𝝲 = 90o
The four types of orthorhombic systems (simple, base centered, face-centered, and body-centered orthorhombic cells) are illustrated below:
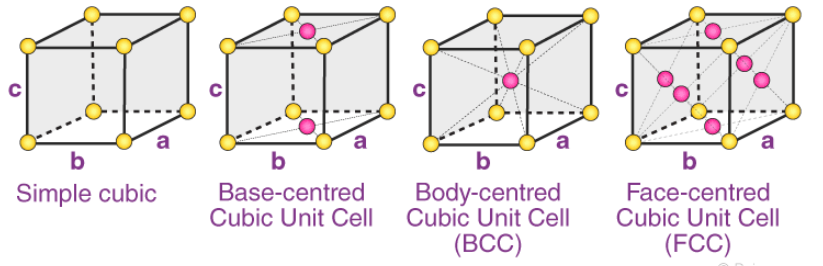
Examples of Orthorhombic Systems:
- Rhombic Sulphur has a simple orthorhombic structure
- Magnesium sulfate heptahydrate (MgSO4.7H2O) is made up of a base centered orthorhombic structure.
- Potassium Nitrate has a structure which is body-centered orthorhombic.
- An example of a substance with a face-centered orthorhombic structure is barium sulfate.
3. Tetragonal Systems
In tetragonal Bravais lattices, the following relations are observed:
a = b ≠ c
𝛂 = 𝞫 = 𝝲 = 90o
The two types of tetragonal systems are simple tetragonal cells and body-centered tetragonal cells, as illustrated below:
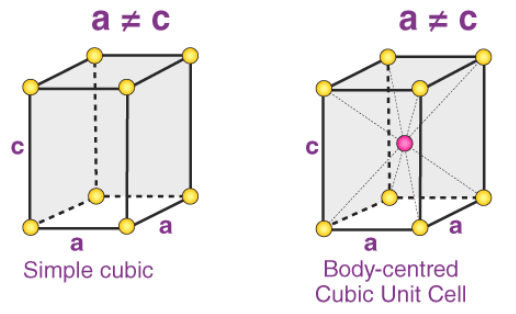
Examples of tetragonal Bravais lattices: Stannic oxide (simple tetragonal) and titanium dioxide (body-centered tetragonal)
4. Monoclinic Systems
Bravais lattices having monoclinic systems obey the following relations:
a ≠ b ≠ c
𝞫 = 𝝲 = 90o and 𝛂 ≠ 90o
The two possible types of monoclinic systems are primitive and base centered monoclinic cells, as illustrated below:
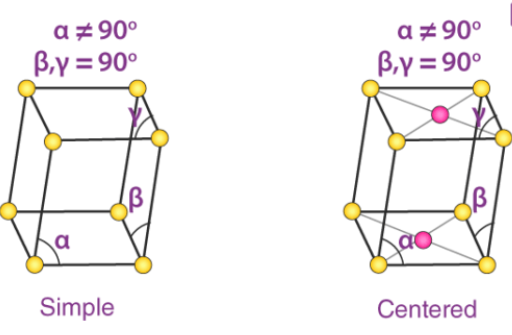
Examples of Monoclinic cells: Monoclinic sulphur (simple monoclinic) and sodium sulfate decahydrate (base centered monoclinic)
5. Triclinic System
There exists only one type of triclinic Bravais lattice, which is a primitive cell. It obeys the following relationship.
a ≠ b ≠ c
𝛂 ≠ 𝞫 ≠ 𝝲 ≠ 90o
An illustration of a simple triclinic cell is given below:
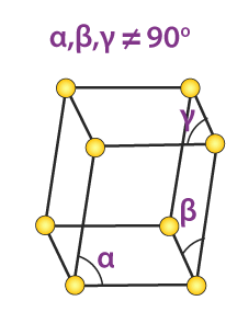
Such unit cells are found in the structure of potassium dichromate (Chemical formula K2Cr2O7).
6. Rhombohedral System
Only the primitive unit cell for a rhombohedral system exists. Its cell relation is given by:
a = b = c
𝛂 = 𝞫 = 𝝲 ≠ 90o
An illustration of the primitive rhombohedral cell is provided below:
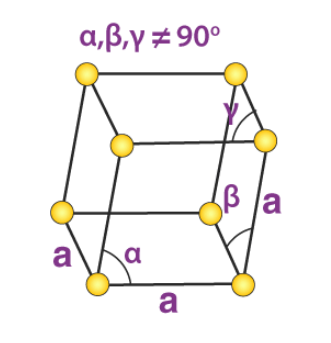
Calcite and sodium nitrate are made up of simple rhombohedral unit cells.
7. Hexagonal System
The only type of hexagonal Bravais lattice is the simple hexagonal cell. It has the following relations between cell sides and angles.
a = b ≠ c
𝛂 = 𝞫 = 90o and 𝝲 = 120o
An illustration of a simple hexagonal cell is provided below:
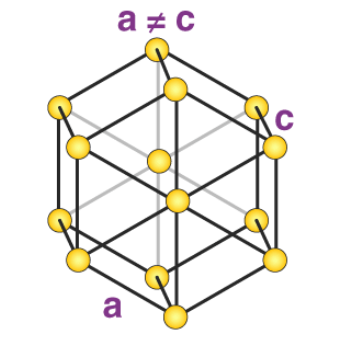
Zinc oxide and beryllium oxide are made up of simple hexagonal unit cells.
Effective Number of atoms in unit cell:
Effective Number of atoms in a unit cell is the most basic unit of a crystal lattice. It is made up of a series of atoms. Crystalline solids are defined by the arrangement of their unit cells, which dictates their structure and symmetry. A component particle is often located at a lattice point in the unit cell, which indicates that it is a component particle. Remember, though, that a lattice point might also be empty.
We must now find out what the effective number of atoms per unit cell is. Let us begin by discussing some of the general characteristics of unit cells and their structure. At different lattice sites, the contribution of atoms varies.
It takes eight unit cells in total to share the space occupied by a particle (atom, molecule, or ion) positioned at the lattice’s four corners. Consequently, the contribution of an atom in the corner to a single unit cell is one-eighth of the total contribution of the atom.
Two unit cells share an atom on the unit cell’s face, which makes them a pair. Consequently, the contribution of a particle near the face to a unit cell is half that of a particle farther away.
The component particle at the edge center of the lattice is shared by four unit cells in the lattice. As a consequence, it makes up one-fourth of the total contribution to any given unit cell.
In the lattice, an atom located in the center of the body of a unit cell is unique to that particular unit cell. There is no exchange of information taking place.
So, let us now calculate the number of component particles present at all times in unit cells at any given moment.
Primitive Cubic Units:
You may be aware that the component particles of a primitive unit cell, whether they be atoms or molecules or ions, can only be located in the corners of the unit
cell. Consequently, the effective number of atoms in a unit cell in the corner of a unit cell contributes one-eighth of itself to the total amount of matter in that unit cell. A cube now has eight corners, which is an improvement.
Consequently, in a primitive unit cell, there are eight particles at each of the cubic structure’s eight corners, for a total of sixteen particles. Because of this, the total contribution may be calculated in the following manner:
1/8 (contribution of corner atoms) × 8 (number of atoms in the corners) = 1
Body Centered Unit Cells (BCC):
Let’s now turn our attention to what the number of atoms in bcc arrangement is? In a body-centered unit cell, there one atom in each of the eight corners and one atom in the center of the cell. It is now possible to distinguish between particles in the center and those on the margins regarding their contributions. The periphery contributes one-eighth of a particular unit cell’s total capacity. The centre unit cell is not shared by any other unit cells in the lattice. The total number of atoms in a body-centered unit cell is therefore determined as follows when we calculate the total number of atoms:
(1/8 × 8) + (1 × 1) = 2
Face Centered Unit Cells (FCC):
Face-Centered unit cell particles are now present throughout the edges and faces of the cubic structure, forming a face-centered unit with cell particles. The atoms in the eight corners each contribute one-eighth of the total amount of energy in the unit cell. When there is a lattice, two unit cells share the atoms at the face of the structure equally. Consequently, their contribution is just a half-atom in size, at most. Note that a cubic cell has six faces, which is important to remember. Thus, the total number of atoms in a face-centered unit cell is
(1/8 × 8) + (1/2 × 6) = 4
NUMBER OF PARTICLES PER UNIT CELL IN A CUBIC CRYSTAL SYSTEM CALCULATION:
1. Calculation of the contribution of each atom at each lattice location
A corner atom is shared by eight unit cells. Hence essentially its contribution is = 1x(1/8) = 1/8.
Because an atom on the face is shared by two unit cells, its contribution equals = 1x(1/2) = 1/2.
An atom at the center of a unit cell is not shared by any other unit cell, its contribution is = 1
An atom on edge is shared by four unit cells, its contribution is = 1x(1/4) = 1/4
2. Counting the number of atoms in a unit cell
Simple [basic] unit cell: It only has eight atoms present in the corners, each of which contributes 1/8, therefore 8 x 1/8 = 1 atom.
Body Centered unit cell (BCC):
1/8 x 8 = 1
1 atom in the middle = 1 x 1 = 1
So the total number of atoms is 1 + 1 = 2 atoms.
Face Centered unit cell (FCC):
Atomic contribution at the corner = 1/8 x 8 = 1
Atomic contribution at faces = 1/2 x 6 = 3
So the total number of atoms is 3 + 1 = 4.
Unit Cell Packing Efficiency:
No matter how densely the component particles, molecules, atoms, or ions are packed, there will always be some space in the form of voids. The packing efficiency of a unit cell refers to how much of the cell is occupied by atoms. Because packing spheres even without any space between them is impossible, the percentage should be less than 100%. As a result, packing efficiency is equal to the packing factor times 100.
The proportion of the total space in a unit cell which is occupied by constituent particles like atoms, ions, or molecules packed within the lattice is described as packing efficiency. In three-dimensional space, packing efficiency is the total amount of space occupied by such particles. Simply said, the fraction of a solid’s total volume that is occupied by spherical atoms may be calculated.
Packing Efficiency in the Face Centered Cubic (FCC) Unit Cell:
A Face Centered Cubic Unit Cell seems to be the most densely occupied unit cell. A single atom can also be discovered at the center of each of the cube’s faces. Because each atom only makes up half of the cell, these face-centered atoms are shared between two adjacent unit cells.
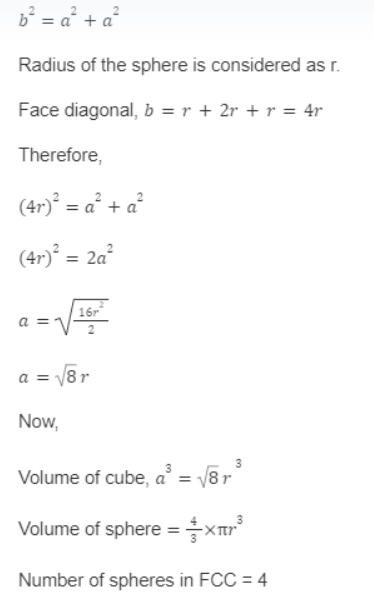
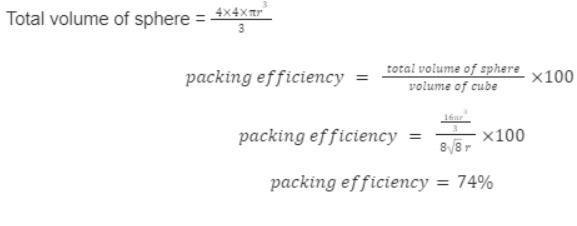
Packing Efficiency in the Body Centered Cubic (BCC) Unit Cell:
Having eight atoms in each corner and one in the middle, the BCC is essentially equal to a basic cubic unit cell. BCC has an open structure. The atom in the centre belongs to the Unit cell where it is found exclusively.
Packing Efficiency of Bcc Unit Cell Formula
Important points regarding calculation of atoms in BCC are as follows:
- 8 (eight) corners generate 1/8 atoms at each corner that is equals to 8 × 1/8 = 1 atom.
- At the center of body, one atom is equal to one atom.
- Two atoms form body-centered cubic unit cell.
Let,
a = edge length
c = body diagonal length
b = length of diagonal
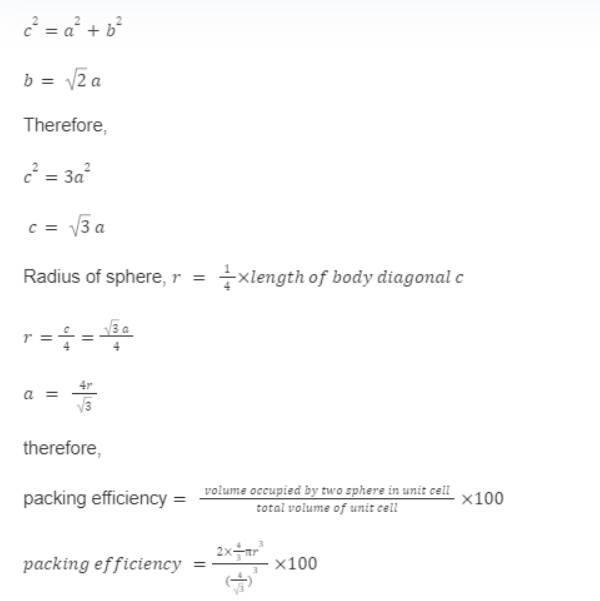
Packing Efficiency = 68%
Packing Efficiency of BCC unit cell is 68%.
Packing Efficiency of Simple Cubic Unit Cell:
r=a/2
a = 2r
Therefore,
Packing Efficiency =volume occupied by one atom total volume of unit cell ×100
Packing Efficiency =43r3 (2r)3 ×100
Packing Efficiency = 52.4%
Packing efficiency of simple cubic unit cell is 52.4%.